The Sun supplies the Earth with some 3.9x10 to the -6 EJ of radiant energy every year. This compares with a global primary energy consumption of 508 EJ and electric power consumption of 62 EJ in 2009.1 The solar resource is thus clearly capable of meeting the current – and projected – human energy consumption many times over, even allowing for moderate conversion efficiency and allowing for the fact that solar energy can be easily harvested only from a fraction of the Earth’s surface.
Until now only a fraction of 1% of energy has been provided by direct conversion of solar energy, but that is set to change: International Energy Agency projections anticipate that some 11% of electric power will be provided by solar technologies by 2050.2
Challenges remain, however, in driving up efficiencies and scalability, driving down costs, in order to ensure that solar energy is a competitive and environmentally friendly energy source for the future.

Solar energy is harvested through the absorption of light in a material. Three different varieties of solar energy conversion may be distinguished: solar thermal conversion where light energy is converted to heat, solar chemical conversion where light energy is converted to stored chemical potential energy, and solar photovoltaic conversion where light energy is converted to electrical energy.
Absorption of a photon of visible light results in the excitation of an electron from its ground state, where it is normally involved in bonding, to a higher energy state where it is freer. That electron could then relax back to its original state, giving up its energy through a series of collisions, generating heat, as is the case in solar thermal technologies. Or it could take part in a chemical reaction and give up its energy to form a new chemical bond. This is what happens in photosynthesis and in the generation of synthetic ‘solar’ fuels.
Alternatively, if the electron is able to travel through the absorbing material towards a contact with an external circuit, taking some of its additional electrochemical energy with it, then it is able to do electrical work. This latter process is the heart of photovoltaic (PV) energy conversion, the direct conversion of radiant light energy to electricity.
Solar Photovoltaic Technology
PV conversion requires a material with a minimum energy threshold for excitation of electrons, necessary to avoid very rapid relaxation of electrons to their ground state. Additionally it requires that the two contacts between the absorbing material and the external circuit be different, in order to provide a preferred direction for the excited electrons to travel.
In the most common type of device, PV conversion is carried out using a large area film of a semiconductor material such as silicon which has been treated (or doped) differently on the top and bottom in order to provide the differences in the electrical contacts. A semiconductor possesses the required energy gap and is sufficiently conductive for electrons and holes (positive charges) to travel easily, while the difference in doping provides the direction.
Some 80% of solar panels are currently made from silicon (Si) wafers processed into this p-n junction device structure and connected together into modules of around 1 square meter in area. Silicon PV panels convert some 15-20% of the incident solar energy into electricity.
Against a picture where an established technology is growing strongly and becoming cost competitive, the following question arises: what can new materials do for solar power?
By 2011, a total of 69 GWp of PV panels were installed worldwide with a capacity to generate some 85TWh of electricity annually.3 The cost of a typical, grid connected PV system is around $3/Wp of which around half is the cost of installation, mounting and electrical regulation, the so-called balance of systems (BoS).4
The cost of PV modules has reduced rapidly over the last 10 years, and especially over the last two years, due to a market expansion partly stimulated by incentives such as feed-in tariffs. At current costs, the price of solar generated electricity is almost competitive with grid power in the sunniest countries5 and in countries where grid power is already expensive6, and it will soon become competitive in other countries as the market continues to expand. In Germany, where peak daily electricity usage coincides with peak PV generation7, the high (25 GWp) installed PV capacity has effectively reduced the price of peak electricity.8
Hence against a picture where an established technology is growing strongly and becoming cost competitive, the following question arises: what can new materials do for solar power?
New Materials for Solar Power
Until the last few years, Si PV was widely seen as too expensive for widespread use. That led first to the development of alternative ‘thin film’ semiconductor materials which could be deposited more cheaply than Si wafers, which absorb light more strongly and so required less material, and which could easily be deposited into integrated module structures eliminating the need for costly processing of individual cells into interconnected modules. Two particular thin film materials, cadmium telluride (CdTe) and copper indium gallium diselenide (CIGS) have been developed commercially and offer module efficiencies of 13% and 16% respectively (cf 18-22% for Si).9 CdTe in particular has been developed with encouragingly low manufacturing costs.4
However, concerns about the availability of rare elements used in these materials (In and Te) and toxicity of materials (such as Cd), as well as the ambition to reduce the cost of PV device manufacturing even further, have led to a surge of research into very different materials. These include efforts to reduce module cost using organic, inorganic and hybrid (organic:inorganic) semiconductor materials that can be processed directly from solution using printing or coating techniques. Solution processed semiconductors can be combined with flexible substrates to make very light weight solar cell materials in varying shapes and colours.
The goal of reducing the cost of PV power has also led to research into materials enabling higher efficiency PV energy conversion. In one high efficiency approach, materials that are sensitive to different parts of the solar spectrum are combined into a so called multi-junction device in order to improve the efficiency of converting different wavelengths of light.
What can these new technologies deliver?
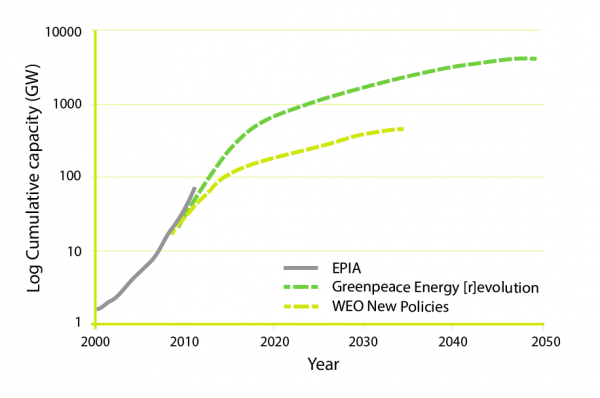
Exploiting the Potential of New Technologies
The original motivation for new photovoltaic materials was to reduce PV power costs by reducing the cost of the modules compared to crystalline silicon. Whilst new materials with cheaper manufacturing costs make this possible, the margin by which power costs can be reduced is becoming narrower, both because of recent reductions in the cost of Si solar cell manufacture and because the module now comprises only around half of the cost of the PV system.
However, there are other ways in which the cost of the PV system could be reduced by using new technologies, for example, by making use of lighter module weight, and by integrating modules into building components so that the cost of mounting structures is reduced.
In minimising the overall cost of the PV system it is important to consider the particular application, location, demand pattern and available resources. A low efficiency, flexible PV technology may be ideal for a large membrane roof or applications in the developing world where local demand is low. On the other hand, an expensive, high efficiency technology may work best under high insolation and clear skies and especially where mechanical components can be used to allow the solar cell to follow the Sun.
New PV technologies can bring other benefits than simply reducing the cost of the PV system, especially in terms of the goal of reducing carbon emissions. One important aspect is how quickly PV capacity can be scaled up to meet the growing demand for low carbon electricity. The graph opposite shows that PV capacity will have to increase by over a factor of 50 by 2050 to meet international projections. Such growth would demand high availability of component materials and a module manufacturing technology that can be rapidly scaled.
11% of electric power will be provided by solar technologies by 2050
These issues have stimulated research into alternatives to rare elements such as In and Te for inorganic thin films and alternative contact metals to silver, and research into in thinner silicon structures. In this context, organic photovoltaic technology has the advantage not only of abundant, synthetically available, hydrocarbon-based raw material but also the widespread availability of plant and know-how in printing and coating capacity that could facilitate rapid scale-up.
However, organic PV is disadvantaged by having much lower module efficiencies (~3%) than commercially available inorganic technologies, as well as having limited module lifetimes of a few years. Nevertheless, organic PV has shown astonishing improvement over the last decade with efficiencies of research cells increasing from 2% to 10%,10 and confidence that 12-15% is achievable. These promising cell efficiencies should soon be translated to modules.
At Imperial College, a large research effort is focussed upon the goal of developing new and better organic and hybrid PV materials. This effort includes the design and synthesis of new semiconducting polymers and molecules, the characterisation of these materials in terms of their light absorption, electrical properties and material structure, the development of deposition processes that allow control of the structure during printing or coating, and the fabrication, measurement and modelling of solar cell devices.
Outstanding challenges in this field are, firstly, knowing how the electrical current generation processes in the material are related to the chemical structure of the materials used, and secondly, determining and controlling the physical structure within the films, and finally, designing semiconductor and contact materials that are stable with regard to chemical and physical degradation.
The amount of energy used in the manufacture of PV systems is another pertinent consideration in the goal to reduce carbon emissions. Whilst conventional PV systems have a carbon intensity of around 45 gCO2 / kWh generated,12 this drops to less than 20 gCO2 / kWh in the case of cheaper, thin film or printed PV technologies.13 14 15
A consequence of this is that in the near term carbon emissions could be reduced more quickly using low carbon intensity PV materials, even if the efficiency is lower. At Imperial College, methods to assess the carbon emissions mitigation potential of new, pre-commercial PV technologies are being developed in order to identify the materials, production processes and applications for maximum impact on carbon emissions reductions.17
Engineering Efficiencies
A final and fundamentally important goal of research into new PV materials is to find ways to improve the power conversion efficiency, not only by improving the quality of the semiconductor materials, contact materials and device structures used in typical solar cells, but also by exploring the fundamental mechanisms of power conversion efficiency. Herein lies the tantalising challenge of finding new ways to harness some of the solar energy that can’t be converted in a conventional device.
In a solar cell made from a single semiconductor material some 70% of incident solar energy is lost due to transmission of photons with energy lower than the absorption threshold of that material and to thermal relaxation of charges generated by photons of higher energy. In principle these losses might be reduced by using materials that absorb either low or high energy photons and re-emit photons of different energy, so effectively changing the shape of the incident spectrum.
Another approach is to exploit the properties of nanoparticulate or molecular semiconductors to slow down the rate at which charges lose energy. A different and still unexplored goal is to combine the functions of photovoltaic and photochemical energy conversion in a single device, so that some solar energy can be chemically stored until needed. Development and understanding of synthetic solar ‘fuels’ that can store solar energy is one of the future goals of solar energy materials research.